Active Plasmonics
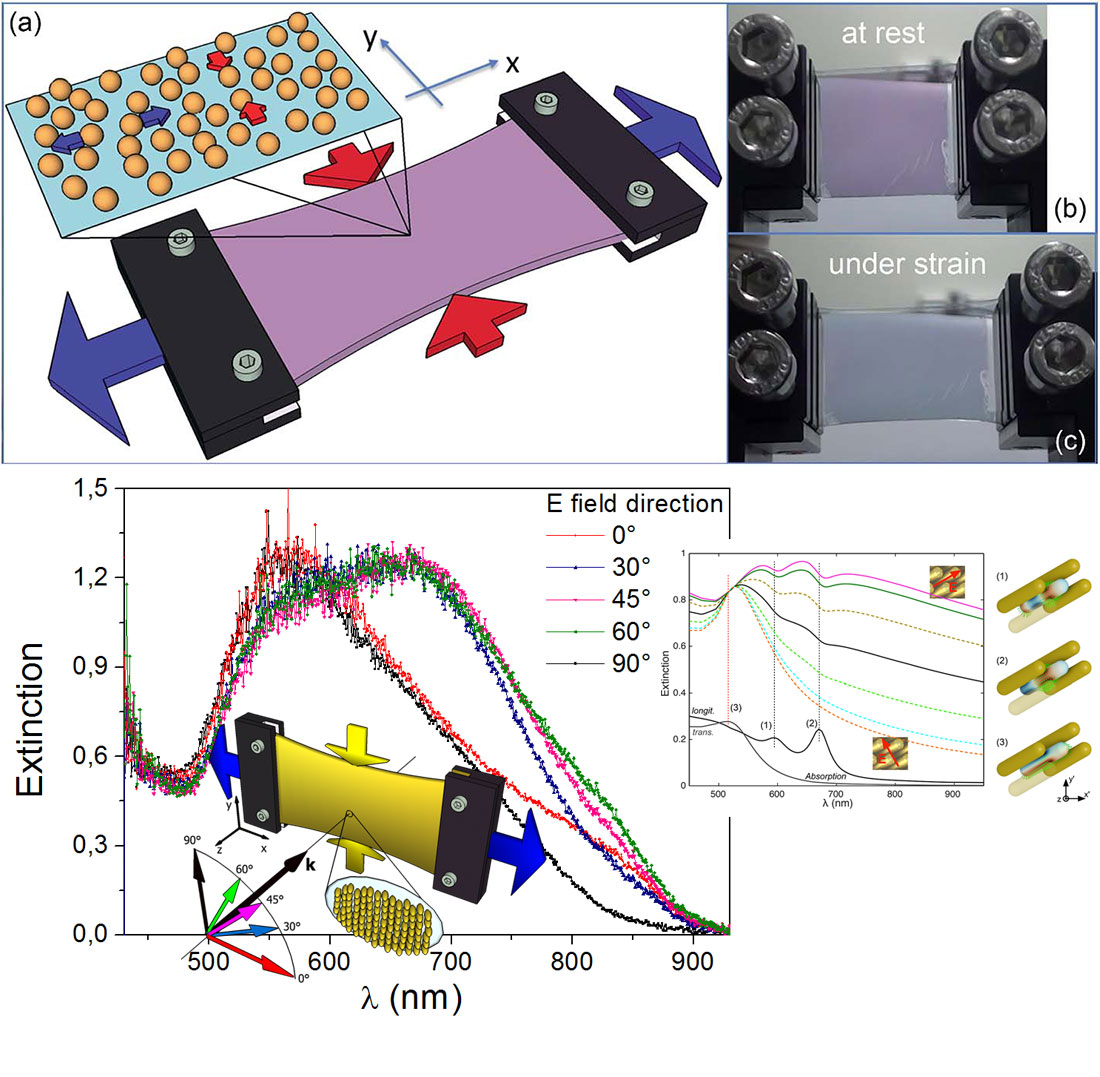
Plasmonic coupling
When nanoparticles are very close to each other, near-field coupling leads to concentrated and highly localized electric fields. Our past and ongoing experimental activities demonstrate that influencing the plasmonic coupling at the nanoscale can be as simple as pulling a rubber stripe. The prototype is made of a PDMS sample where a single layer of Au nanoparticles where immobilized by electrostatic interaction (collaboration with Prof. Buergi, Univ. of Geneva). By stretching the sample, the average distance between the nanoparticles becomes larger in the stretching direction and shorter in the perpendicular one, giving rise to a visible change of colour. This technology can be efficiently exploited for deformation sensing. Many other more complex possibilities can be envisioned like nanoscale platforms for biological or biomedical experiments.
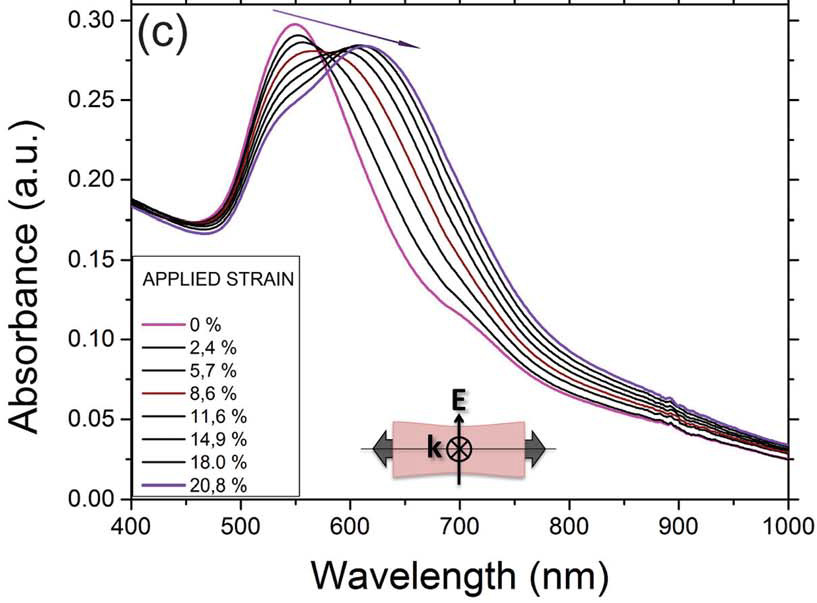
Surrounding medium effect
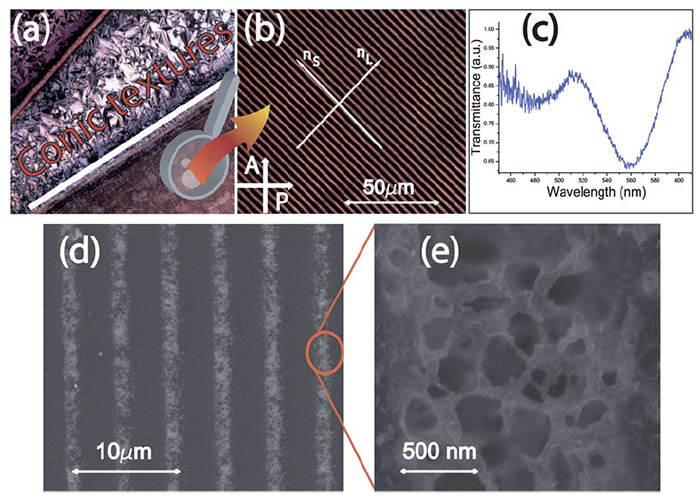
Collective plasmon resonances
Gratings of metal nanoparticles have been investigated for their optical properties since the beginning of the 1990s. Compared to their isolated counterparts, which support localized plasmon modes, those gratings present “lattice plasmon modes” which are dispersive and moreover exhibit in their optical spectra very narrow features which can take the shape of sharp maxima or minima. In regular arrays, these features arise when an “edge- diffraction” occurs for a wavelength close to localized surface plasmon resonances. Edge diffraction happens at so-called Rayleigh wavelengths, when one of the diffracted order changes from radiative (shorter wavelength) to evanescent (longer wavelength). The transition corresponds to grazing propagation of the diffracted order along the grating, and the perpendicular component of the wavevector cancels. In most of the theoretical and experimental configurations, such systems have been investigated at normal incidence, where the Rayleigh wavelengths solely depend on the period of the grating. However, as the edge diffraction depends as well on the incidence angle of the illumination, playing with the direction of the incoming plane wave gives the advantage to tune the position of the associated features. The fact that the lineshapes of spectra around Rayleigh wavelengths can be very narrow makes those kind of systems very suitable for designing plasmon nanolasers, or for biosensing applications as the associated figures of merit are expected to be large. For the same reason, they could be used for monitoring of mechanical stress and deformations.
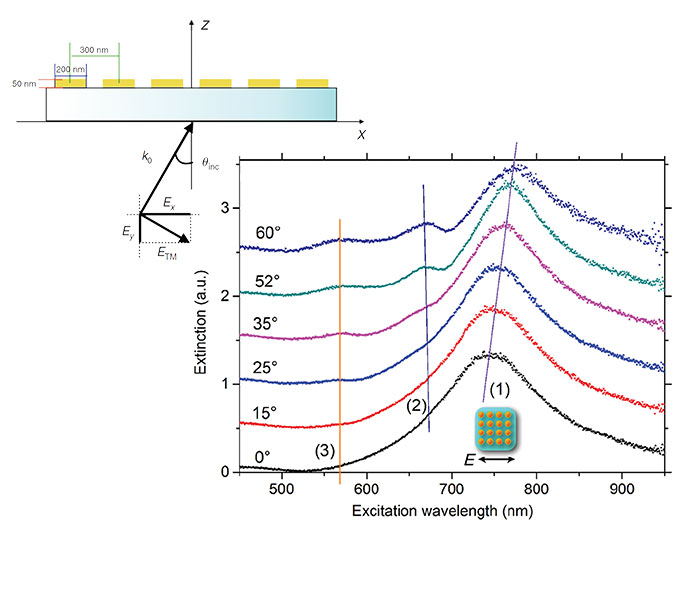